The Knot Series #3: DNA Visuazation and Modeling
Learn how scientists use knot theory to visualize and model the topological structure of DNA to understand the effect of different biological conditions
Sunskritha R Shivaprasad
10/13/20245 min read
DNA Visualization and Modeling:
The molecule that carries the blueprint for life, DNA, has complex structural behavior. As it carries out essential tasks in the cell, it frequently gets knotted, supercoiled, or entangled. A special lens through which to view and comprehend these dynamic DNA configurations is provided by knot theory, a branch of mathematics that was initially created to investigate loops and entanglements. Scientists have learned a great deal about how DNA folds, twists, and untangles itself to ensure effective genetic regulation and replication by putting the concepts of knot theory to use.
1. DNA Supercoiling and Topology: A Mathematical Perspective on Genetic Twists
The study of DNA topology—how DNA strands twist, coil, and occasionally knot as they carry out tasks like transcription and replication—is one of the most well-known biological applications of knot theory. The DNA helix can become supercoiled or tangled as a result of the mechanical stress it experiences in small cellular spaces, especially in bacteria, which have circular DNA. The enzymes that must function to keep the DNA in its functional state may encounter difficulties as a result of these tangles and twists.
This is where knot theory comes into play. DNA topology is controlled by enzymes such as topoisomerases, which cut, rejoin, or untangle the strands as needed. In order to model how these enzymes break up knots and supercoils and keep DNA from becoming too twisted to work, knot theory offers a mathematical framework. Scientists may also predict how enzymes will interact with DNA in certain situations by modeling the topological structures of DNA. This improved comprehension enables scientists to see the tangles and knots in real time, demonstrating how supercoiling affects the availability of genetic material for transcription, replication, and other vital biological functions.
Modeling DNA knots and supercoiling has made it possible to create useful drugs, particularly for the treatment of cancer. Topoisomerases are the target of several cancer medications, which stop them from untangling DNA during cell division and cause cancer cells to die. Researchers can develop more potent medications that can specifically target these enzymes and enhance patient outcomes by using knot theory and DNA topology studies.
2. DNA Knots and Supercoiling
Because the DNA helix must unwind and relieve torsional strain during activities like transcription and replication, DNA supercoiling naturally occurs. However, this strain frequently results in supercoiling, a process where DNA coils over itself, in densely populated cellular settings. Furthermore, under stress or during recombination processes, some circular DNA structures, like bacterial plasmids, may knot.
Knot theory treats DNA as a flexible string-like material that can bend, twist, and create knots, enabling researchers to quantify these patterns. Scientists can forecast the topological complexity of these knots and supercoils using mathematical models, which aids in their comprehension of the ways in which these structures impact molecular processes. They are able to determine, for instance, how tightly wrapped DNA is or how many times a DNA strand crosses over itself. These models are crucial for illustrating the limitations that DNA encounters during gene expression, cell division, and recombination.
3. Chromosomal Structure: Chromosome Conformation Capture
Knot theory covers the larger-scale folding and arrangement of chromosomes in addition to the study of individual DNA strands. The long, densely packed DNA molecules called chromosomes, which are present in the nucleus of eukaryotic cells, fold extensively to fit into the small area while preserving the accessibility of genes for transcription and replication. These chromosomes are not arranged randomly; rather, the topological characteristics of the DNA have an impact.
Scientists may map the three-dimensional folding patterns of chromosomes within the cell by using knot theory to analyze Chromosome Conformation Capture (3C) data. These models aid scientists in comprehending the potential effects of chromosomal twists, knots, and loops on gene regulation. For example, a chromosomal knot can facilitate interaction by bringing distant genes and regulatory elements closer together. However, chromosomal instability may result from these knots' capacity to obstruct cell division.
4. Progressing with DNA Visualization: Novel Approaches to Knot Theory
Exciting new methods for viewing DNA activity under various settings are being produced by the ongoing application of knot theory to DNA research. Recent developments in imaging techniques, such as atomic force microscopy (AFM) and nanopore technology, have made it possible for researchers to watch live how DNA coils, twists, and knots itself. When combined with knot theory's mathematical models, these results provide a more realistic picture of how DNA changes dynamically in various biological settings. For instance, knot theory has clarified the mechanisms behind DNA replication and recombination, especially in circular molecules such as bacterial plasmids.
See you in November!
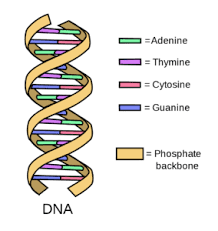
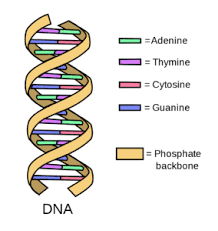
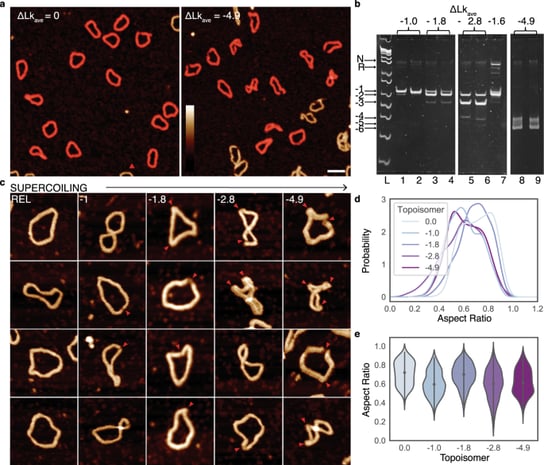
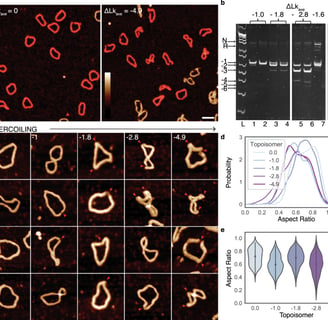
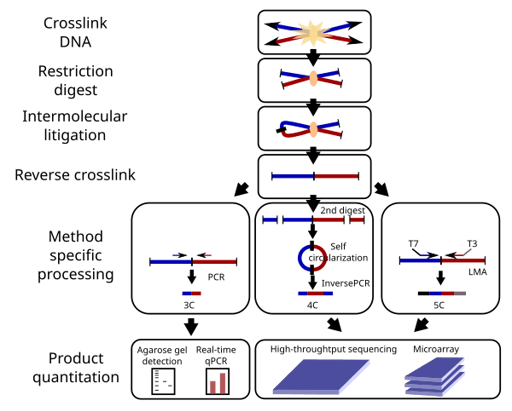
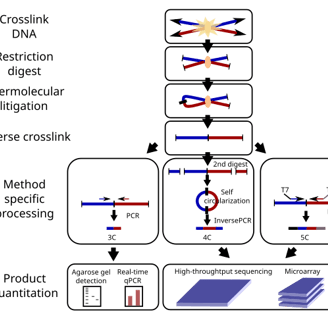
Technology used in Chromosome conformation capture used to model the topology of chromosomes
3C techniques consist of:
4C: A technique that concentrates on just one locus
5C: A technique that looks for interaction between every piece in a certain area
Hi-C: An objective method that finds interactions between every possible piece
Capture-C: A modular strategy that enriches for relevant interactions using oligonucleotides
Hi-C: A focused method that enhances a library's collection of sequences of interest before sequencing
By using this topological method, scientists are starting to understand how chromosomal arrangement affects biological functions like DNA repair, gene expression, and even aging.
a. DNA Minicircle Images (AFM):
Atomic force microscopy (AFM) images show that as DNA becomes more negatively supercoiled (twisted in the opposite direction of its helix), it compacts into tighter, twisted shapes. Individual circles of DNA (highlighted in red) were isolated from these images for closer analysis.
b. Gel Visualization of DNA Compaction:
A gel (like a specialized Jell-O) was used to display different shapes of DNA minicircles. These shapes depend on the level of twisting, from “nicked” (a relaxed, untwisted form) to highly compacted forms (produced by adding ethidium bromide to lock in these shapes). Each band on the gel represents a different degree of DNA compaction.
c. Compaction and Structural Changes of Minicircles:
Images of DNA minicircles at various twisting levels show that more twisted minicircles become highly compact and develop "kinks" or bends in their structure (marked by red arrows). These changes highlight how DNA’s shape adjusts under pressure.
d. Relationship Between DNA Shape and Twisting (KDE Plot):
This plot shows the likelihood of finding a certain DNA shape depending on how much it’s twisted. It essentially maps the "shape diversity" of DNA minicircles for each twist level.
e. Violin Plot of DNA Compaction Levels:
A violin plot is used to show how much DNA minicircles compact based on their twist. It provides a visual distribution of each DNA shape for different levels of supercoiling, helping scientists compare how DNA tightens up under strain.
Structure of a coiled double-stranded DNA
Contacts
sunskrithas19@gmail.com
Blog
© TheArtGene
sunskrithas@theartgene.com
Subscribe to our blog!
Updates bi-weekly every Sunday!